Molecular cation and low-dimensional perovskite surface passivation in perovskite solar cells – Nature.com
Abstract
The deposition of large ammonium cations onto perovskite surfaces to passivate defects and reduce contact recombination has enabled exceptional efficiency and stability in perovskite solar cells. These ammonium cations can either assemble as a thin molecular layer at the perovskite surface or induce the formation of a low-dimensional (usually two-dimensional) perovskite capping layer on top of the three-dimensional perovskite. The formation of these two different structures is often overlooked by researchers, although they impact differently on device operation. In this Review, we seek to distinguish between these two passivation layers. We consider the conditions needed for the formation of low-dimensional perovskite and the electronic properties of the two structures. We discuss the mechanisms by which each method improves photovoltaic efficiency and stability. Finally, we summarize the knowledge gaps that need to be addressed to better understand and optimize ammonium cation-based passivation strategies.
This is a preview of subscription content, access via your institution
Access options
style{display:none!important}.LiveAreaSection-193358632 *{align-content:stretch;align-items:stretch;align-self:auto;animation-delay:0s;animation-direction:normal;animation-duration:0s;animation-fill-mode:none;animation-iteration-count:1;animation-name:none;animation-play-state:running;animation-timing-function:ease;azimuth:center;backface-visibility:visible;background-attachment:scroll;background-blend-mode:normal;background-clip:borderBox;background-color:transparent;background-image:none;background-origin:paddingBox;background-position:0 0;background-repeat:repeat;background-size:auto auto;block-size:auto;border-block-end-color:currentcolor;border-block-end-style:none;border-block-end-width:medium;border-block-start-color:currentcolor;border-block-start-style:none;border-block-start-width:medium;border-bottom-color:currentcolor;border-bottom-left-radius:0;border-bottom-right-radius:0;border-bottom-style:none;border-bottom-width:medium;border-collapse:separate;border-image-outset:0s;border-image-repeat:stretch;border-image-slice:100%;border-image-source:none;border-image-width:1;border-inline-end-color:currentcolor;border-inline-end-style:none;border-inline-end-width:medium;border-inline-start-color:currentcolor;border-inline-start-style:none;border-inline-start-width:medium;border-left-color:currentcolor;border-left-style:none;border-left-width:medium;border-right-color:currentcolor;border-right-style:none;border-right-width:medium;border-spacing:0;border-top-color:currentcolor;border-top-left-radius:0;border-top-right-radius:0;border-top-style:none;border-top-width:medium;bottom:auto;box-decoration-break:slice;box-shadow:none;box-sizing:border-box;break-after:auto;break-before:auto;break-inside:auto;caption-side:top;caret-color:auto;clear:none;clip:auto;clip-path:none;color:initial;column-count:auto;column-fill:balance;column-gap:normal;column-rule-color:currentcolor;column-rule-style:none;column-rule-width:medium;column-span:none;column-width:auto;content:normal;counter-increment:none;counter-reset:none;cursor:auto;display:inline;empty-cells:show;filter:none;flex-basis:auto;flex-direction:row;flex-grow:0;flex-shrink:1;flex-wrap:nowrap;float:none;font-family:initial;font-feature-settings:normal;font-kerning:auto;font-language-override:normal;font-size:medium;font-size-adjust:none;font-stretch:normal;font-style:normal;font-synthesis:weight style;font-variant:normal;font-variant-alternates:normal;font-variant-caps:normal;font-variant-east-asian:normal;font-variant-ligatures:normal;font-variant-numeric:normal;font-variant-position:normal;font-weight:400;grid-auto-columns:auto;grid-auto-flow:row;grid-auto-rows:auto;grid-column-end:auto;grid-column-gap:0;grid-column-start:auto;grid-row-end:auto;grid-row-gap:0;grid-row-start:auto;grid-template-areas:none;grid-template-columns:none;grid-template-rows:none;height:auto;hyphens:manual;image-orientation:0deg;image-rendering:auto;image-resolution:1dppx;ime-mode:auto;inline-size:auto;isolation:auto;justify-content:flexStart;left:auto;letter-spacing:normal;line-break:auto;line-height:normal;list-style-image:none;list-style-position:outside;list-style-type:disc;margin-block-end:0;margin-block-start:0;margin-bottom:0;margin-inline-end:0;margin-inline-start:0;margin-left:0;margin-right:0;margin-top:0;mask-clip:borderBox;mask-composite:add;mask-image:none;mask-mode:matchSource;mask-origin:borderBox;mask-position:0 0;mask-repeat:repeat;mask-size:auto;mask-type:luminance;max-height:none;max-width:none;min-block-size:0;min-height:0;min-inline-size:0;min-width:0;mix-blend-mode:normal;object-fit:fill;object-position:50% 50%;offset-block-end:auto;offset-block-start:auto;offset-inline-end:auto;offset-inline-start:auto;opacity:1;order:0;orphans:2;outline-color:initial;outline-offset:0;outline-style:none;outline-width:medium;overflow:visible;overflow-wrap:normal;overflow-x:visible;overflow-y:visible;padding-block-end:0;padding-block-start:0;padding-bottom:0;padding-inline-end:0;padding-inline-start:0;padding-left:0;padding-right:0;padding-top:0;page-break-after:auto;page-break-before:auto;page-break-inside:auto;perspective:none;perspective-origin:50% 50%;pointer-events:auto;position:static;quotes:initial;resize:none;right:auto;ruby-align:spaceAround;ruby-merge:separate;ruby-position:over;scroll-behavior:auto;scroll-snap-coordinate:none;scroll-snap-destination:0 0;scroll-snap-points-x:none;scroll-snap-points-y:none;scroll-snap-type:none;shape-image-threshold:0;shape-margin:0;shape-outside:none;tab-size:8;table-layout:auto;text-align:initial;text-align-last:auto;text-combine-upright:none;text-decoration-color:currentcolor;text-decoration-line:none;text-decoration-style:solid;text-emphasis-color:currentcolor;text-emphasis-position:over right;text-emphasis-style:none;text-indent:0;text-justify:auto;text-orientation:mixed;text-overflow:clip;text-rendering:auto;text-shadow:none;text-transform:none;text-underline-position:auto;top:auto;touch-action:auto;transform:none;transform-box:borderBox;transform-origin:50% 50%0;transform-style:flat;transition-delay:0s;transition-duration:0s;transition-property:all;transition-timing-function:ease;vertical-align:baseline;visibility:visible;white-space:normal;widows:2;width:auto;will-change:auto;word-break:normal;word-spacing:normal;word-wrap:normal;writing-mode:horizontalTb;z-index:auto;-webkit-appearance:none;-moz-appearance:none;-ms-appearance:none;appearance:none;margin:0}.LiveAreaSection-193358632{width:100%}.LiveAreaSection-193358632 .login-option-buybox{display:block;width:100%;font-size:17px;line-height:30px;color:#222;padding-top:30px;font-family:Harding,Palatino,serif}.LiveAreaSection-193358632 .additional-access-options{display:block;font-weight:700;font-size:17px;line-height:30px;color:#222;font-family:Harding,Palatino,serif}.LiveAreaSection-193358632 .additional-login>li:not(:first-child)::before{transform:translateY(-50%);content:””;height:1rem;position:absolute;top:50%;left:0;border-left:2px solid #999}.LiveAreaSection-193358632 .additional-login>li:not(:first-child){padding-left:10px}.LiveAreaSection-193358632 .additional-login>li{display:inline-block;position:relative;vertical-align:middle;padding-right:10px}.BuyBoxSection-683559780{display:flex;flex-wrap:wrap;flex:1;flex-direction:row-reverse;margin:-30px -15px 0}.BuyBoxSection-683559780 .box-inner{width:100%;height:100%;padding:30px 5px;display:flex;flex-direction:column;justify-content:space-between}.BuyBoxSection-683559780 p{margin:0}.BuyBoxSection-683559780 .readcube-buybox{background-color:#f3f3f3;flex-shrink:1;flex-grow:1;flex-basis:255px;background-clip:content-box;padding:0 15px;margin-top:30px}.BuyBoxSection-683559780 .subscribe-buybox{background-color:#f3f3f3;flex-shrink:1;flex-grow:4;flex-basis:300px;background-clip:content-box;padding:0 15px;margin-top:30px}.BuyBoxSection-683559780 .subscribe-buybox-nature-plus{background-color:#f3f3f3;flex-shrink:1;flex-grow:4;flex-basis:100%;background-clip:content-box;padding:0 15px;margin-top:30px}.BuyBoxSection-683559780 .title-readcube,.BuyBoxSection-683559780 .title-buybox{display:block;margin:0;margin-right:10%;margin-left:10%;font-size:24px;line-height:32px;color:#222;text-align:center;font-family:Harding,Palatino,serif}.BuyBoxSection-683559780 .title-asia-buybox{display:block;margin:0;margin-right:5%;margin-left:5%;font-size:24px;line-height:32px;color:#222;text-align:center;font-family:Harding,Palatino,serif}.BuyBoxSection-683559780 .asia-link{color:#069;cursor:pointer;text-decoration:none;font-size:1.05em;font-family:-apple-system,BlinkMacSystemFont,”Segoe UI”,Roboto,Oxygen-Sans,Ubuntu,Cantarell,”Helvetica Neue”,sans-serif;line-height:1.05em6}.BuyBoxSection-683559780 .access-readcube{display:block;margin:0;margin-right:10%;margin-left:10%;font-size:14px;color:#222;padding-top:10px;text-align:center;font-family:-apple-system,BlinkMacSystemFont,”Segoe UI”,Roboto,Oxygen-Sans,Ubuntu,Cantarell,”Helvetica Neue”,sans-serif;line-height:20px}.BuyBoxSection-683559780 ul{margin:0}.BuyBoxSection-683559780 .link-usp{display:list-item;margin:0;margin-left:20px;padding-top:6px;list-style-position:inside}.BuyBoxSection-683559780 .link-usp span{font-size:14px;color:#222;font-family:-apple-system,BlinkMacSystemFont,”Segoe UI”,Roboto,Oxygen-Sans,Ubuntu,Cantarell,”Helvetica Neue”,sans-serif;line-height:20px}.BuyBoxSection-683559780 .access-asia-buybox{display:block;margin:0;margin-right:5%;margin-left:5%;font-size:14px;color:#222;padding-top:10px;text-align:center;font-family:-apple-system,BlinkMacSystemFont,”Segoe UI”,Roboto,Oxygen-Sans,Ubuntu,Cantarell,”Helvetica Neue”,sans-serif;line-height:20px}.BuyBoxSection-683559780 .access-buybox{display:block;margin:0;margin-right:10%;margin-left:10%;font-size:14px;color:#222;opacity:.8px;padding-top:10px;text-align:center;font-family:-apple-system,BlinkMacSystemFont,”Segoe UI”,Roboto,Oxygen-Sans,Ubuntu,Cantarell,”Helvetica Neue”,sans-serif;line-height:20px}.BuyBoxSection-683559780 .price-buybox{display:block;font-size:30px;color:#222;font-family:-apple-system,BlinkMacSystemFont,”Segoe UI”,Roboto,Oxygen-Sans,Ubuntu,Cantarell,”Helvetica Neue”,sans-serif;padding-top:30px;text-align:center}.BuyBoxSection-683559780 .price-buybox-to{display:block;font-size:30px;color:#222;font-family:-apple-system,BlinkMacSystemFont,”Segoe UI”,Roboto,Oxygen-Sans,Ubuntu,Cantarell,”Helvetica Neue”,sans-serif;text-align:center}.BuyBoxSection-683559780 .price-info-text{font-size:16px;padding-right:10px;color:#222;font-family:-apple-system,BlinkMacSystemFont,”Segoe UI”,Roboto,Oxygen-Sans,Ubuntu,Cantarell,”Helvetica Neue”,sans-serif}.BuyBoxSection-683559780 .price-value{font-size:30px;font-family:-apple-system,BlinkMacSystemFont,”Segoe UI”,Roboto,Oxygen-Sans,Ubuntu,Cantarell,”Helvetica Neue”,sans-serif}.BuyBoxSection-683559780 .price-per-period{font-family:-apple-system,BlinkMacSystemFont,”Segoe UI”,Roboto,Oxygen-Sans,Ubuntu,Cantarell,”Helvetica Neue”,sans-serif}.BuyBoxSection-683559780 .price-from{font-size:14px;padding-right:10px;color:#222;font-family:-apple-system,BlinkMacSystemFont,”Segoe UI”,Roboto,Oxygen-Sans,Ubuntu,Cantarell,”Helvetica Neue”,sans-serif;line-height:20px}.BuyBoxSection-683559780 .issue-buybox{display:block;font-size:13px;text-align:center;color:#222;font-family:-apple-system,BlinkMacSystemFont,”Segoe UI”,Roboto,Oxygen-Sans,Ubuntu,Cantarell,”Helvetica Neue”,sans-serif;line-height:19px}.BuyBoxSection-683559780 .no-price-buybox{display:block;font-size:13px;line-height:18px;text-align:center;padding-right:10%;padding-left:10%;padding-bottom:20px;padding-top:30px;color:#222;font-family:-apple-system,BlinkMacSystemFont,”Segoe UI”,Roboto,Oxygen-Sans,Ubuntu,Cantarell,”Helvetica Neue”,sans-serif}.BuyBoxSection-683559780 .vat-buybox{display:block;margin-top:5px;margin-right:20%;margin-left:20%;font-size:11px;color:#222;padding-top:10px;padding-bottom:15px;text-align:center;font-family:-apple-system,BlinkMacSystemFont,”Segoe UI”,Roboto,Oxygen-Sans,Ubuntu,Cantarell,”Helvetica Neue”,sans-serif;line-height:17px}.BuyBoxSection-683559780 .tax-buybox{display:block;width:100%;color:#222;padding:20px 16px;text-align:center;font-family:-apple-system,BlinkMacSystemFont,”Segoe UI”,Roboto,Oxygen-Sans,Ubuntu,Cantarell,”Helvetica Neue”,sans-serif;line-height:NaNpx}.BuyBoxSection-683559780 .button-container{display:flex;padding-right:20px;padding-left:20px;justify-content:center}.BuyBoxSection-683559780 .button-container>*{flex:1px}.BuyBoxSection-683559780 .button-container>a:hover,.Button-505204839:hover,.Button-1078489254:hover,.Button-2737859108:hover{text-decoration:none}.BuyBoxSection-683559780 .btn-secondary{background:#fff}.BuyBoxSection-683559780 .button-asia{background:#069;border:1px solid #069;border-radius:0;cursor:pointer;display:block;padding:9px;outline:0;text-align:center;text-decoration:none;min-width:80px;margin-top:75px}.BuyBoxSection-683559780 .button-label-asia,.ButtonLabel-3869432492,.ButtonLabel-3296148077,.ButtonLabel-1636778223{display:block;color:#fff;font-size:17px;line-height:20px;font-family:-apple-system,BlinkMacSystemFont,”Segoe UI”,Roboto,Oxygen-Sans,Ubuntu,Cantarell,”Helvetica Neue”,sans-serif;text-align:center;text-decoration:none;cursor:pointer}.Button-505204839,.Button-1078489254,.Button-2737859108{background:#069;border:1px solid #069;border-radius:0;cursor:pointer;display:block;padding:9px;outline:0;text-align:center;text-decoration:none;min-width:80px;max-width:320px;margin-top:20px}.Button-505204839 .btn-secondary-label,.Button-1078489254 .btn-secondary-label,.Button-2737859108 .btn-secondary-label{color:#069}
/* style specs end */
Access Nature and 54 other Nature Portfolio journals
Get Nature+, our best-value online-access subscription
$29.99 / 30 days
cancel any time
Subscribe to this journal
Receive 12 digital issues and online access to articles
$119.00 per year
only $9.92 per issue
Buy this article
- Purchase on Springer Link
- Instant access to full article PDF
Prices may be subject to local taxes which are calculated during checkout
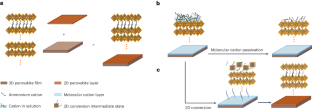
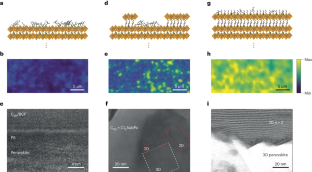
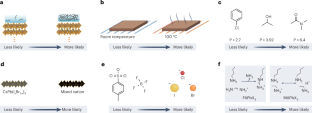
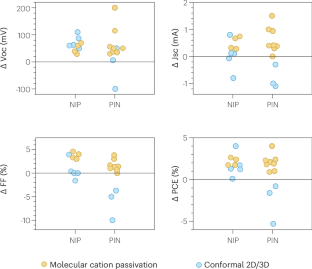
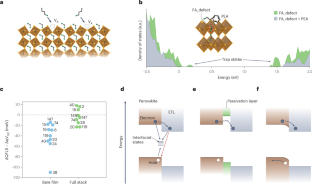
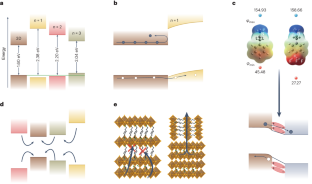
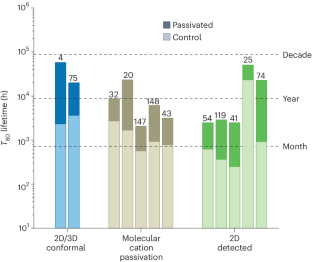
References
-
Chen, H. et al. Improved charge extraction in inverted perovskite solar cells with dual-site-binding ligands. Science 384, 189–193 (2024).
-
Jiang, Q. et al. Surface passivation of perovskite film for efficient solar cells. Nat. Photon. 13, 460–466 (2019).
-
Jang, Y. W. et al. Intact 2D/3D halide junction perovskite solar cells via solid-phase in-plane growth. Nat. Energy 6, 63–71 (2021).
-
Sidhik, S. et al. Deterministic fabrication of 3D/2D perovskite bilayer stacks for durable and efficient solar cells. Science 377, 1425–1430 (2022).
-
Proppe, A. H. et al. Multication perovskite 2D/3D interfaces form via progressive dimensional reduction. Nat. Commun. 12, 3472 (2021).
-
Ugur, E. et al. Front-contact passivation through 2D/3D perovskite heterojunctions enables efficient bifacial perovskite/silicon tandem solar cells. Matter 6, 2919–2934 (2023).
-
Quan, L. N. et al. Ligand-stabilized reduced-dimensionality perovskites. J. Am. Chem. Soc. 138, 2649–2655 (2016).
-
Li, X., Hoffman, J. M. & Kanatzidis, M. G. The 2D halide perovskite rulebook: how the spacer influences everything from the structure to optoelectronic device efficiency. Chem. Rev. 121, 2230–2291 (2021).
-
Xue, J. et al. Reconfiguring the band-edge states of photovoltaic perovskites by conjugated organic cations. Science 371, 636–640 (2021).
-
Zhao, T., Chueh, C.-C., Chen, Q., Rajagopal, A. & Jen, A. K.-Y. Defect passivation of organic–inorganic hybrid perovskites by diammonium iodide toward high-performance photovoltaic devices. ACS Energy Lett. 1, 757–763 (2016).
-
Tan, S. et al. Stability-limiting heterointerfaces of perovskite photovoltaics. Nature 605, 268–273 (2022).
-
Caiazzo, A. et al. 3D perovskite passivation with a benzotriazole-based 2D interlayer for high-efficiency solar cells. ACS Appl. Energy Mater. 6, 3933–3943 (2023).
-
Gharibzadeh, S. et al. Record open‐circuit voltage wide‐bandgap perovskite solar cells utilizing 2D/3D perovskite heterostructure. Adv. Energy Mater. 9, 1803699 (2019).
-
Lai, H. et al. High-performance flexible all-perovskite tandem solar cells with reduced VOC-deficit in wide-bandgap subcell. Adv. Energy Mater. 12, 2202438 (2022).
-
Degani, M. et al. 23.7% Efficient inverted perovskite solar cells by dual interfacial modification. Sci. Adv. 7, 7930 (2021).
-
Cacovich, S. et al. Imaging and quantifying non-radiative losses at 23% efficient inverted perovskite solar cells interfaces. Nat. Commun. 13, 2868 (2022).
-
Xia, J. et al. Deep surface passivation for efficient and hydrophobic perovskite solar cells. J. Mater. Chem. A Mater. 9, 2919–2927 (2021).
-
Hu, H. et al. Perovskite quantum wells formation mechanism for stable efficient perovskite photovoltaics—a real‐time phase‐transition study. Adv. Mater. 33, 2006238 (2021).
-
Kodalle, T. et al. Revealing the transient formation dynamics and optoelectronic properties of 2D Ruddlesden–Popper phases on 3D perovskites. Adv. Energy Mater. 13, 2201490 (2023).
-
Park, S. M. et al. Engineering ligand reactivity enables high-temperature operation of stable perovskite solar cells. Science 381, 209–215 (2023).
-
Chakkamalayath, J., Hiott, N. & Kamat, P. V. How stable is the 2D/3D interface of metal halide perovskite under light and heat? ACS Energy Lett. 8, 169–171 (2023).
-
Yoo, J. J. et al. An interface stabilized perovskite solar cell with high stabilized efficiency and low voltage loss. Energy Environ. Sci. 12, 2192–2199 (2019).
-
Suo, J. et al. Interfacial engineering from material to solvent: a mechanistic understanding on stabilizing α-formamidinium lead triiodide perovskite photovoltaics. Nano Energy 94, 106924 (2022).
-
Chen, H. et al. Quantum-size-tuned heterostructures enable efficient and stable inverted perovskite solar cells. Nat. Photon. 16, 352–358 (2022).
-
Zhao, X. et al. Accelerated aging of all-inorganic, interface-stabilized perovskite solar cells. Science 377, 307–310 (2022).
-
Juarez-Perez, E. J., Ono, L. K. & Qi, Y. Thermal degradation of formamidinium based lead halide perovskites into sym-triazine and hydrogen cyanide observed by coupled thermogravimetry-mass spectrometry analysis. J. Mater. Chem. A Mater. 7, 16912–16919 (2019).
-
Brunetti, B., Cavallo, C., Ciccioli, A., Gigli, G. & Latini, A. On the thermal and thermodynamic (in)stability of methylammonium lead halide perovskites. Sci. Rep. 6, 31896 (2016).
-
Li, T. et al. Inorganic wide-bandgap perovskite subcells with dipole bridge for all-perovskite tandems. Nat. Energy 8, 610–620 (2023).
-
Steele, J. A. et al. Thermal unequilibrium of strained black CsPbI3 thin films. Science 365, 679–684 (2019).
-
Kerner, R. A. et al. Amine additive reactions induced by the soft Lewis acidity of Pb2+ in halide perovskites. Part I: evidence for Pb–alkylamide formation. J. Mater. Chem. C Mater. 7, 5251–5259 (2019).
-
Li, Z. et al. Ammonia for post-healing of formamidinium-based perovskite films. Nat. Commun. 13, 4417 (2022).
-
Jiang, Q. et al. Surface reaction for efficient and stable inverted perovskite solar cells. Nature 611, 278–283 (2022).
-
Yin, W.-J. et al. Unique properties of halide perovskites as possible origins of the superior solar cell performance. Adv. Mater. 26, 4653–4658 (2014).
-
Oner, S. M. et al. Surface defect formation and passivation in formamidinium lead triiodide (FAPbI3) perovskite solar cell absorbers. J. Phys. Chem. Lett. 13, 324–330 (2022).
-
Ross, R. T. Some thermodynamics of photochemical systems. J. Chem. Phys. 46, 4590–4593 (1967).
-
Stolterfoht, M. et al. The impact of energy alignment and interfacial recombination on the internal and external open-circuit voltage of perovskite solar cells. Energy Environ. Sci. 12, 2778–2788 (2019).
-
Warby, J. et al. Understanding performance limiting interfacial recombination in pin perovskite solar cells. Adv. Energy Mater. 12, 2103567 (2022).
-
Chen, H. et al. Regulating surface potential maximizes voltage in all-perovskite tandems. Nature 613, 676–681 (2022).
-
Liu, J. et al. Efficient and stable perovskite–silicon tandem solar cells through contact displacement by MgFx. Science 377, 302–306 (2022).
-
Sutanto, A. A. et al. 2D/3D perovskite engineering eliminates interfacial recombination losses in hybrid perovskite solar cells. Chem 7, 1903–1916 (2021).
-
Azmi, R. et al. Damp heat-stable perovskite solar cells with tailored-dimensionality 2D/3D heterojunctions. Science 376, 73–77 (2022).
-
Liu, C. et al. Tuning structural isomers of phenylenediammonium to afford efficient and stable perovskite solar cells and modules. Nat. Commun. 12, 6394 (2021).
-
Ansari, F. et al. Passivation mechanism exploiting surface dipoles affords high-performance perovskite solar cells. J. Am. Chem. Soc. 142, 11428–11433 (2020).
-
Zhao, L. et al. Chemical polishing of perovskite surface enhances photovoltaic performances. J. Am. Chem. Soc. 144, 1700–1708 (2022).
-
Zhang, Z. et al. Carrier transport through the ultrathin silicon-oxide layer in tunnel oxide passivated contact (TOPCon) c-Si solar cells. Sol. Energy Mater. Sol. Cells 187, 113–122 (2018).
-
Peibst, R. et al. Working principle of carrier selective poly-Si/c-Si junctions: is tunnelling the whole story? Sol. Energy Mater. Sol. Cells 158, 60–67 (2016).
-
Stolterfoht, M. et al. Visualization and suppression of interfacial recombination for high-efficiency large-area pin perovskite solar cells. Nat. Energy 3, 847–854 (2018).
-
Yang, S. et al. Stabilizing halide perovskite surfaces for solar cell operation with wide-bandgap lead oxysalts. Science 365, 473–478 (2019).
-
Wang, Q., Dong, Q., Li, T., Gruverman, A. & Huang, J. Thin insulating tunneling contacts for efficient and water-resistant perovskite solar cells. Adv. Mater. 28, 6734–6739 (2016).
-
Liang, C. et al. Two-dimensional Ruddlesden–Popper layered perovskite solar cells based on phase-pure thin films. Nat. Energy 6, 38–45 (2020).
-
Proppe, A. H. et al. Synthetic control over quantum well width distribution and carrier migration in low-dimensional perovskite photovoltaics. J. Am. Chem. Soc. 140, 2890–2896 (2018).
-
Westbrook, R. J. E. et al. 2D phase purity determines charge-transfer yield at 3D/2D lead halide perovskite heterojunctions. J. Phys. Chem. Lett. 12, 3312–3320 (2021).
-
Green, M. A. The passivated emitter and rear cell (PERC): from conception to mass production. Sol. Energy Mater. Sol. Cells 143, 190–197 (2015).
-
Zhang, F. et al. Metastable Dion–Jacobson 2D structure enables efficient and stable perovskite solar cells. Science 375, 71–76 (2022).
-
Bertoluzzi, L. et al. Mobile ion concentration measurement and open-access band diagram simulation platform for halide perovskite solar cells. Joule 4, 109–127 (2020).
-
Eames, C. et al. Ionic transport in hybrid lead iodide perovskite solar cells. Nat. Commun. 6, 7497 (2015).
-
Thiesbrummel, J. et al. Universal current losses in perovskite solar cells due to mobile ions. Adv. Energy Mater. 11, 2101447 (2021).
-
Gutierrez-Partida, E. et al. Large-grain double cation perovskites with 18 μs lifetime and high luminescence yield for efficient inverted perovskite solar cells. ACS Energy Lett. 6, 1045–1054 (2021).
-
Dasgupta, A. et al. Visualizing macroscopic inhomogeneities in perovskite solar cells. ACS Energy Lett. 7, 2311–2322 (2022).
-
Chen, C. et al. Arylammonium-assisted reduction of the open-circuit voltage deficit in wide-bandgap perovskite solar cells: the role of suppressed ion migration. ACS Energy Lett. 5, 2560–2568 (2020).
-
Teale, S. et al. Dimensional mixing increases the efficiency of 2D/3D perovskite solar cells. J. Phys. Chem. Lett. 11, 5115–5119 (2020).
-
Liu, Z. et al. In situ observation of vapor-assisted 2D–3D heterostructure formation for stable and efficient perovskite solar cells.Nano Lett. 20, 1296–1304 (2020).
-
Lin, D. et al. Stable and scalable 3D–2D planar heterojunction perovskite solar cells via vapor deposition. Nano Energy 59, 619–625 (2019).
-
Dualeh, A. et al. Effect of annealing temperature on film morphology of organic–inorganic hybrid pervoskite solid-state solar cells. Adv. Funct. Mater. 24, 3250–3258 (2014).
-
La-Placa, M. G. et al. Vacuum-deposited 2D/3D perovskite heterojunctions. ACS Energy Lett. 4, 2893–2901 (2019).
-
Smith, I. C., Hoke, E. T., Solis-Ibarra, D., McGehee, M. D. & Karunadasa, H. I. A layered hybrid perovskite solar-cell absorber with enhanced moisture stability. Ang. Chem. Int. Ed. 53, 11232–11235 (2014).
-
Emery, Q. et al. Encapsulation and outdoor testing of perovskite solar cells: comparing industrially relevant process with a simplified lab procedure. ACS Appl. Mater. Interfaces 14, 5159–5167 (2022).
-
Zhu, H. et al. Long-term operating stability in perovskite photovoltaics. Nat. Rev. Mater. 8, 569–586 (2023).
-
Brinkmann, K. O. et al. Suppressed decomposition of organometal halide perovskites by impermeable electron-extraction layers in inverted solar cells. Nat. Commun. 8, 13938 (2017).
-
Hoffmann, L. et al. Spatial atmospheric pressure atomic layer deposition of tin oxide as an impermeable electron extraction layer for perovskite solar cells with enhanced thermal stability. ACS Appl. Mater. Interfaces 10, 6006–6013 (2018).
-
Domanski, K. et al. Not all that glitters is gold: metal-migration-induced degradation in perovskite solar cells. ACS Nano 10, 6306–6314 (2016).
-
Lin, Y. et al. Suppressed ion migration in low-dimensional perovskites. ACS Energy Lett. 2, 1571–1572 (2017).
-
Huang, Z. et al. Suppressed ion migration in reduced-dimensional perovskites improves operating stability. ACS Energy Lett. 4, 1521–1527 (2019).
-
Chen, R. et al. Reduction of bulk and surface defects in inverted methylammonium- and bromide-free formamidinium perovskite solar cells. Nat. Energy 8, 839–849 (2023).
-
Luo, L. et al. Stabilization of 3D/2D perovskite heterostructures via inhibition of ion diffusion by cross-linked polymers for solar cells with improved performance. Nat. Energy 8, 294–303 (2023).
-
Sutanto, A. A. et al. Dynamical evolution of the 2D/3D interface: a hidden driver behind perovskite solar cell instability. J. Mater. Chem. A Mater. 8, 2343–2348 (2020).
-
Cho, J., Dubose, J. T., Le, A. N. T. & Kamat, P. V. Suppressed halide ion migration in 2D lead halide perovskites. ACS Mater. Lett. 2, 565–570 (2020).
-
Wang, M. et al. Ammonium cations with high pKa in perovskite solar cells for improved high-temperature photostability. Nat. Energy 8, 1229–1239 (2023).
-
Yang, W. S. et al. High-performance photovoltaic perovskite layers fabricated through intramolecular exchange. Science 348, 1234–1237 (2015).
-
Jiang, Q. et al. Enhanced electron extraction using SnO2 for high-efficiency planar-structure HC(NH2)2PbI3-based perovskite solar cells. Nat. Energy 2, 16177 (2017).
-
Bi, D. et al. Polymer-templated nucleation and crystal growth of perovskite films for solar cells with efficiency greater than 21%. Nat. Energy 1, 16142 (2016).
-
Tan, H. et al. Efficient and stable solution-processed planar perovskite solar cells via contact passivation. Science 355, 722–726 (2017).
-
Zheng, X. et al. Defect passivation in hybrid perovskite solar cells using quaternary ammonium halide anions and cations. Nat. Energy 2, 17102 (2017).
-
Cui, P. et al. Planar p–n homojunction perovskite solar cells with efficiency exceeding 21.3%. Nat. Energy 4, 150–159 (2019).
-
Jeon, N. J. et al. A fluorene-terminated hole-transporting material for highly efficient and stable perovskite solar cells. Nat. Energy 3, 682–689 (2018).
-
Jung, E. H. et al. Efficient, stable and scalable perovskite solar cells using poly(3-hexylthiophene). Nature 567, 511–515 (2019).
-
Yang, D. et al. High efficiency planar-type perovskite solar cells with negligible hysteresis using EDTA-complexed SnO2. Nat. Commun. 9, 3239 (2018).
-
Wang, L. et al. A Eu3+-Eu2+ ion redox shuttle imparts operational durability to Pb-I perovskite solar cells. Science 363, 265–270 (2019).
-
Li, N. et al. Cation and anion immobilization through chemical bonding enhancement with fluorides for stable halide perovskite solar cells. Nat. Energy 4, 408–415 (2019).
-
Chen, Y. et al. Impacts of alkaline on the defects property and crystallization kinetics in perovskite solar cells. Nat. Commun. 10, 1112 (2019).
-
Zhu, C. et al. Strain engineering in perovskite solar cells and its impacts on carrier dynamics. Nat. Commun. 10, 815 (2019).
-
Kim, M. et al. Methylammonium chloride induces intermediate phase stabilization for efficient perovskite solar cells. Joule 3, 2179–2192 (2019).
-
Chung, J. et al. Impact of electrode materials on process environmental stability of efficient perovskite solar cells. Joule 3, 1977–1985 (2019).
-
Xu, Z. et al. A thermodynamically favored crystal orientation in mixed formamidinium/methylammonium perovskite for efficient solar cells. Adv. Mater. 31, 1900390 (2019).
-
Niu, X. et al. Temporal and spatial pinhole constraints in small-molecule hole transport layers for stable and efficient perovskite photovoltaics. J. Mater. Chem. A Mater. 7, 7338–7346 (2019).
-
Kim, H. et al. Optimal interfacial engineering with different length of alkylammonium halide for efficient and stable perovskite solar cells. Adv. Energy Mater. 9, 1902740 (2019).
-
Abuhelaiqa, M. et al. Stable perovskite solar cells using tin acetylacetonate based electron transporting layers. Energy Environ. Sci. 12, 1910–1917 (2019).
-
Zheng, X. et al. Managing grains and interfaces via ligand anchoring enables 22.3%-efficiency inverted perovskite solar cells. Nat. Energy 5, 131–140 (2020).
-
Xie, J. et al. Perovskite bifunctional device with improved electroluminescent and photovoltaic performance through interfacial energy-band engineering. Adv. Mater. 31, 1902543 (2019).
-
Hang, P. et al. An interlayer with strong Pb–Cl bond delivers ultraviolet-filter-free, efficient, and photostable perovskite solar cells. iScience 21, 217–227 (2019).
-
Wang, P. et al. Gradient energy alignment engineering for planar perovskite solar cells with efficiency over 23%. Adv. Mater. 32, 1905766 (2020).
-
Zhang, L. et al. Side-chain engineering on dopant-free hole-transporting polymers toward highly efficient perovskite solar cells (20.19%). Adv. Funct. Mater. 29, 1904856 (2019).
-
Zhang, Y. et al. Achieving reproducible and high-efficiency (>21%) perovskite solar cells with a presynthesized FAPbI3 powder. ACS Energy Lett. 5, 360–366 (2020).
-
Min, H. et al. Efficient, stable solar cells by using inherent bandgap of α-phase formamidinium lead iodide. Science 366, 749–753 (2019).
-
Yoo, J. J. et al. Efficient perovskite solar cells via improved carrier management. Nature 590, 587–593 (2021).
-
Peng, J. et al. Centimetre-scale perovskite solar cells with fill factors of more than 86 per cent. Nature 601, 573–578 (2022).
-
Wu, S. et al. 2D metal–organic framework for stable perovskite solar cells with minimized lead leakage. Nat. Nanotechnol. 15, 934–940 (2020).
-
Shen, C. et al. Stabilizing formamidinium lead iodide perovskite by sulfonyl-functionalized phenethylammonium salt via crystallization control and surface passivation. Sol. RRL 4, 2000069 (2020).
-
Kim, G. et al. Impact of strain relaxation on performance of α-formamidinium lead iodide perovskite solar cells. Science 370, 108–112 (2020).
-
Kim, M. et al. Conformal quantum dot-SnO2 layers as electron transporters for efficient perovskite solar cells. Science 375, 302–306 (2022).
-
Jeong, J. et al. Pseudo-halide anion engineering for α-FAPbI3 perovskite solar cells. Nature 592, 381–385 (2021).
-
Park, B. et al. Stabilization of formamidinium lead triiodide α-phase with isopropylammonium chloride for perovskite solar cells. Nat. Energy 6, 419–428 (2021).
-
Chen, S., Xiao, X., Gu, H. & Huang, J. Iodine reduction for reproducible and high-performance perovskite solar cells and modules. Sci. Adv. 7, eabe8130 (2021).
-
Min, H. et al. Perovskite solar cells with atomically coherent interlayers on SnO2 electrodes. Nature 598, 444–450 (2021).
-
Li, F. et al. Regulating surface termination for efficient inverted perovskite solar cells with greater than 23% efficiency. J. Am. Chem. Soc. 142, 20134–20142 (2020).
-
Li, N. et al. Liquid medium annealing for fabricating durable perovskite solar cells with improved reproducibility. Science 373, 561–567 (2021).
-
Jeong, M. et al. Stable perovskite solar cells with efficiency exceeding 24.8% and 0.3-V voltage loss. Science 369, 1615–1620 (2020).
-
Zhang, T. et al. Ion-modulated radical doping of spiro-OMeTAD for more efficient and stable perovskite solar cells. Science 377, 495–501 (2022).
-
Yang, G. et al. Stable and low-photovoltage-loss perovskite solar cells by multifunctional passivation. Nat. Photon. 15, 681–689 (2021).
-
Wang, T. et al. Transporting holes stably under iodide invasion in efficient perovskite solar cells. Science 377, 1227–1232 (2022).
-
Li, Z. et al. Organometallic-functionalized interfaces for highly efficient inverted perovskite solar cells. Science 376, 416–420 (2022).
-
Park, J. et al. Controlled growth of perovskite layers with volatile alkylammonium chlorides. Nature 616, 724–730 (2023).
-
Zhao, Y. et al. Inactive (PbI2)2RbCl stabilizes perovskite films for efficient solar cells. Science 377, 531–534 (2022).
-
Li, X. et al. Constructing heterojunctions by surface sulfidation for efficient inverted perovskite solar cells. Science 375, 434–437 (2022).
-
Li, G. et al. Highly efficient p–i–n perovskite solar cells that endure temperature variations. Science 379, 399–403 (2023).
-
Bai, Y. et al. Initializing film homogeneity to retard phase segregation for stable perovskite solar cells. Science 378, 747–754 (2022).
-
Chen, T. et al. Inhibition of defect-induced α-to-δ phase transition for efficient and stable formamidinium perovskite solar cells. Nat. Commun. 14, 6125 (2023).
-
Ye, S. et al. Expanding the low-dimensional interface engineering toolbox for efficient perovskite solar cells. Nat. Energy 8, 284–293 (2023).
-
Li, H. et al. 2D/3D heterojunction engineering at the buried interface towards high-performance inverted methylammonium-free perovskite solar cells. Nat. Energy 8, 946–955 (2023).
-
Li, F. et al. Hydrogen-bond-bridged intermediate for perovskite solar cells with enhanced efficiency and stability. Nat. Photon. 17, 478–484 (2023).
-
Shi, P. et al. Oriented nucleation in formamidinium perovskite for photovoltaics. Nature 620, 323–327 (2023).
-
Peng, W. et al. Reducing nonradiative recombination in perovskite solar cells with a porous insulator contact. Science 379, 683–690 (2023).
-
Liu, X. et al. Perovskite solar cells based on spiro-OMeTAD stabilized with an alkylthiol additive. Nat. Photon. 17, 96–105 (2022).
-
Xu, J. et al. Anion optimization for bifunctional surface passivation in perovskite solar cells. Nat. Mater. 22, 1507–1514 (2023).
-
Yan, L. et al. Fabrication of perovskite solar cells in ambient air by blocking perovskite hydration with guanabenz acetate salt. Nat. Energy 8, 1158–1167 (2023).
-
Li, Z. et al. Stabilized hole-selective layer for high-performance inverted p–i–n perovskite solar cells. Science 382, 284–289 (2023).
-
Zhang, S. et al. Minimizing buried interfacial defects for efficient inverted perovskite solar cells. Science 380, 404–409 (2023).
-
Liu, C. et al. Bimolecularly passivated interface enables efficient and stable inverted perovskite solar cells. Science 382, 810–815 (2023).
-
Park, S. M. et al. Low-loss contacts on textured substrates for inverted perovskite solar cells. Nature 624, 289–294 (2023).
-
Liang, Z. et al. Homogenizing out-of-plane cation composition in perovskite solar cells. Nature 624, 557–563 (2023).
-
Huang, Z. et al. Anion–π interactions suppress phase impurities in FAPbI3 solar cells. Nature 623, 531–537 (2023).
-
Chen, W. et al. Interfacial stabilization for inverted perovskite solar cells with long-term stability. Sci. Bull. 66, 991–1002 (2021).
-
Zhang, T. et al. Stable and efficient 3D–2D perovskite–perovskite planar heterojunction solar cell without organic hole transport layer. Joule 2, 2706–2721 (2018).
-
Madhavan, V. E. et al. CuSCN as hole transport material with 3D/2D perovskite solar cells. ACS Appl. Energy Mater. 3, 114–121 (2020).
-
Hu, S. et al. Optimized carrier extraction at interfaces for 23.6% efficient tin–lead perovskite solar cells. Energy Environ. Sci. 15, 2096–2107 (2022).
-
Zhao, S. et al. General nondestructive passivation by 4-fluoroaniline for perovskite solar cells with improved performance and stability. Small 14, e1803350 (2018).
-
Ye, F. et al. Overcoming C60-induced interfacial recombination in inverted perovskite solar cells by electron-transporting carborane. Nat. Commun. 13, 7454 (2022).
-
You, S. et al. Bifunctional hole-shuttle molecule for improved interfacial energy level alignment and defect passivation in perovskite solar cells. Nat. Energy 8, 515–525 (2023).
-
Zhu, H. et al. Tailored amphiphilic molecular mitigators for stable perovskite solar cells with 23.5% efficiency. Adv. Mater. 32, 1907757 (2020).
-
Lin, R. et al. All-perovskite tandem solar cells with improved grain surface passivation. Nature 603, 73–78 (2022).
Acknowledgements
We acknowledge: the HY-NANO project, which received funding from a European Research Council Starting Grant 2018 grant under the European Union’s Horizon 2020 research and innovation programme (grant agreement number 802862); the SPIKE project, which received funding from a European Research Council Proof of Concept 2022 grant under the European Union’s Horizon 2020 research and innovation programme (grant agreement number 101068936). G.G. acknowledges the GOPV project (CSEAA_00011), which received funds from Bando Ricerca di Sistema—CSEA—TIPO A Piano triennale 2019–2021 Decreto direttoriale 27 Ottobre 2021 del Ministero della Transizione Ecologica; MASE-(ex MITE); and Ministero dell’Università e della Ricerca and University of Pavia through the programme Dipartimenti di Eccellenza 2023–2027. This research was made possible by a US Department of the Navy Office of Naval Research Grant (N00014-20-1-2572). This work was supported in part by the Ontario Research Fund’s Research Excellence programme (ORF7; Ministry of Research and Innovation, Ontario Research Fund; Research Excellence Round 7). S.T. was supported by the Hatch Scholarship for Sustainable Energy Research.
Author information
Authors and Affiliations
Contributions
M.D. and S.T. performed the literature review and contributed equally to writing the paper. B.C. contributed to discussion of the idea and revision of the manuscript. E.H.S. and G.G. conceived of the idea and supervised the work.
Corresponding authors
Ethics declarations
Competing interests
The authors declare no competing interests.
Peer review
Peer review information
Nature Energy thanks Yi-Bing Cheng and the other, anonymous, reviewer(s) for their contribution to the peer review of this work.
Additional information
Publisher’s note Springer Nature remains neutral with regard to jurisdictional claims in published maps and institutional affiliations.
Supplementary information
Supplementary Data 1
Database of 50 articles that investigate the use of 2D perovskite or molecular cation passivation strategies. The database includes the paper title, reference to published article, the ligand (usually a cation) and anion (if applicable) of the passivation treatment, the annealing condition, the 3D perovskite composition, the determined surface structure, the polarity of the device, the effect on Voc, Jsc, FF and PCE of the passivation treatment, stability data and the effect of passivation on quasi-Fermi level splitting.
Source data
Source Data Fig. 1
Database of single junction perovskite solar cell efficiency certifications from 2015 onwards. The database includes full device parameters, references to published articles and whether the cell used an ammonium cation (2D or molecular cation) passivation step.
Source Data Fig. 5
Source data for Fig. 5 (derived from Supplementary Data 1).
Source Data Fig. 6
Source data for Fig. 6c (derived from Supplementary Data 1).
Source Data Fig. 8
Source data for Fig. 8 (derived from Supplementary Data 1).
Rights and permissions
Springer Nature or its licensor (e.g. a society or other partner) holds exclusive rights to this article under a publishing agreement with the author(s) or other rightsholder(s); author self-archiving of the accepted manuscript version of this article is solely governed by the terms of such publishing agreement and applicable law.
About this article
Cite this article
Teale, S., Degani, M., Chen, B. et al. Molecular cation and low-dimensional perovskite surface passivation in perovskite solar cells.
Nat Energy (2024). https://doi.org/10.1038/s41560-024-01529-3
-
Received: 22 February 2023
-
Accepted: 22 March 2024
-
Published: 04 July 2024
-
DOI: https://doi.org/10.1038/s41560-024-01529-3